Going on a hike with a petrologist or mineralogist often takes longer than expected.
They peer into cliff faces and riverbeds to find hints about our planet’s ancient past. They pick up seemingly mundane rocks and use them as the main characters in tales of mountain ranges, continental shifts, and planet formation. To them, each rock tells a story. But the rocks you might find on a hike are only the beginning.
At the Earth and Planets Laboratory (EPL), we study rocks and minerals to understand the origin and dynamic evolution of Earth and planetary interiors—from their crusts to their cores—and the processes that lead to surfaces capable of supporting life. We use a broad range of field-based, lab-based and theory-based tools to explore the physics and chemistry of planetary materials from the atomic to the planetary scale. Using our state-of-the-art high-pressure laboratories, we investigate the minerals, rocks, and melts that make up planetary interiors through a combination of observations from natural samples, high pressure and temperature experiments, computational modeling, and data analysis.
In this article, our petrologists, mineralogists, and mineral physicists have highlighted six questions that we’re working on so that we can better tell the story of our planet.
1) How do life and surface rocks co-evolve through deep time?
2) How does a planet initially obtain, retain or lose its volatile elements like water and carbon dioxide?
3) What phases hold and transport volatile elements in deep Earth cycling?
4) How do volatile elements dissolve in silicate melts?
5) How do we relate seismology to interior composition?
6) How is heat transported in the deep mantle?
1) How do life and surface rocks co-evolve through deep time?
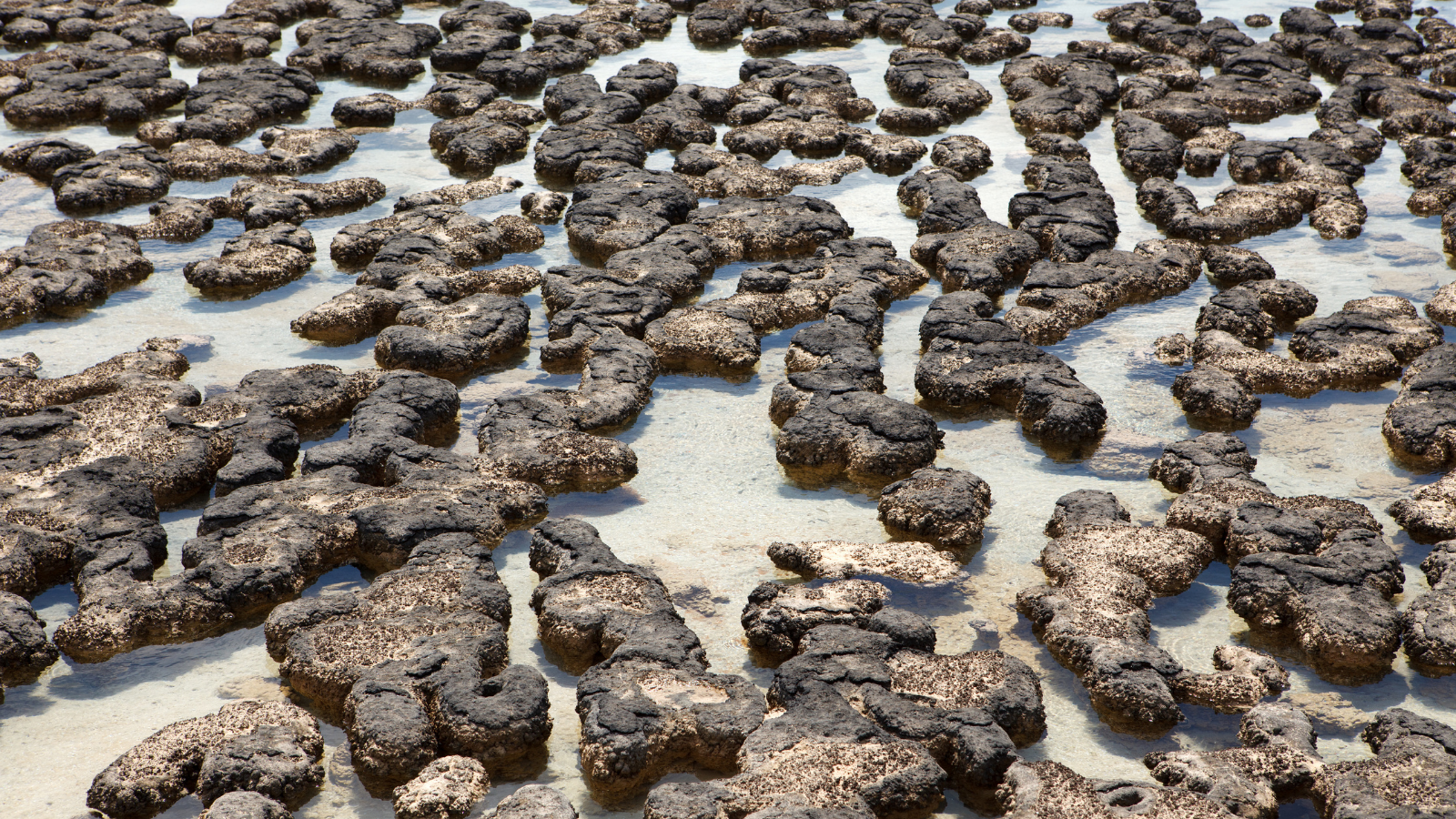
For more than a century, the study of rocks and the minerals that form them has been a focus of Carnegie research. And for most of that hundred-plus years, biology seemed to have nothing whatsoever to do with Earth’s diverse non-living materials. That mindset began to change in the 1990s when a group of Geophysical Laboratory scientists considered possible roles of minerals in life’s chemical origins. And it didn’t take long for them to realize that rocks and life have been intertwined for the past 4 billion years of Earth history: minerals played key roles in life’s origins, and life has returned the favor as one of the most important influences in the formation of minerals. We now understand that the geosphere and biosphere have co-evolved.
How are minerals and life linked?
The realization that minerals and life are linked came into sharp focus in a 2008 paper, “Mineral evolution,” by a team of 8 authors, including 6 current and former Carnegie scientists. That paper and scores of subsequent publications explore the idea that the diversity and distribution of Earth’s minerals (with almost 6000 different “species” known today), has changed dramatically as a consequence of new chemical, physical, and ultimately biological processes. Among the surprising discoveries:
- Most of Earth’s minerals occur because of the pervasive influence of water, which dissolves and concentrates many different chemical elements and thus plays roles in precipitating new minerals and altering old ones.
- Almost half of Earth’s minerals arose because of biological influences, most importantly the rise of atmospheric oxygen through photosynthesis by algae.
- Earth is by far the most mineralogically diverse world in our solar system – a mineralogical richness that may be a unique sign of a living planet.
How can data science help solve Earth science problems?
The study of mineral evolution and the co-evolving geosphere and biosphere continues to be a dynamic field of study for EPL scientists. Notably, Robert Hazen and Shaunna Morrison are developing a new “Evolutionary System” of mineralogy that is attempting to classify all ~6000 minerals according to how they form as a planet evolves. This effort requires the development of large open-access mineral databases and the application of powerful methods of data analysis and visualization to previously hidden relationships between life and rocks, thus moving mineral science into the age of data science.
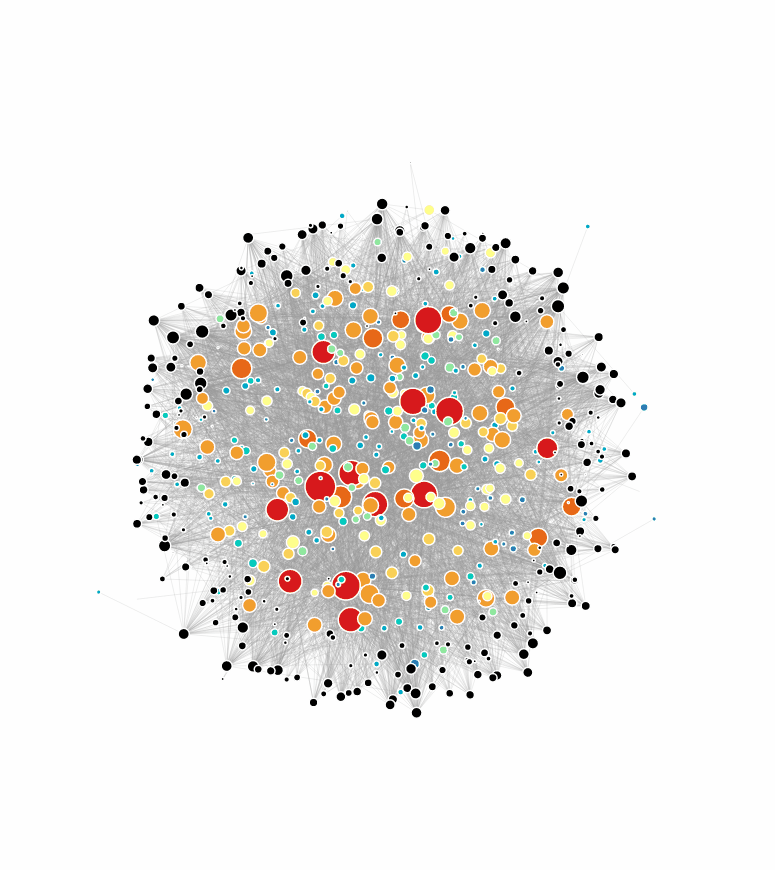
2) How does a planet initially obtain, retain, or lose its volatile elements?
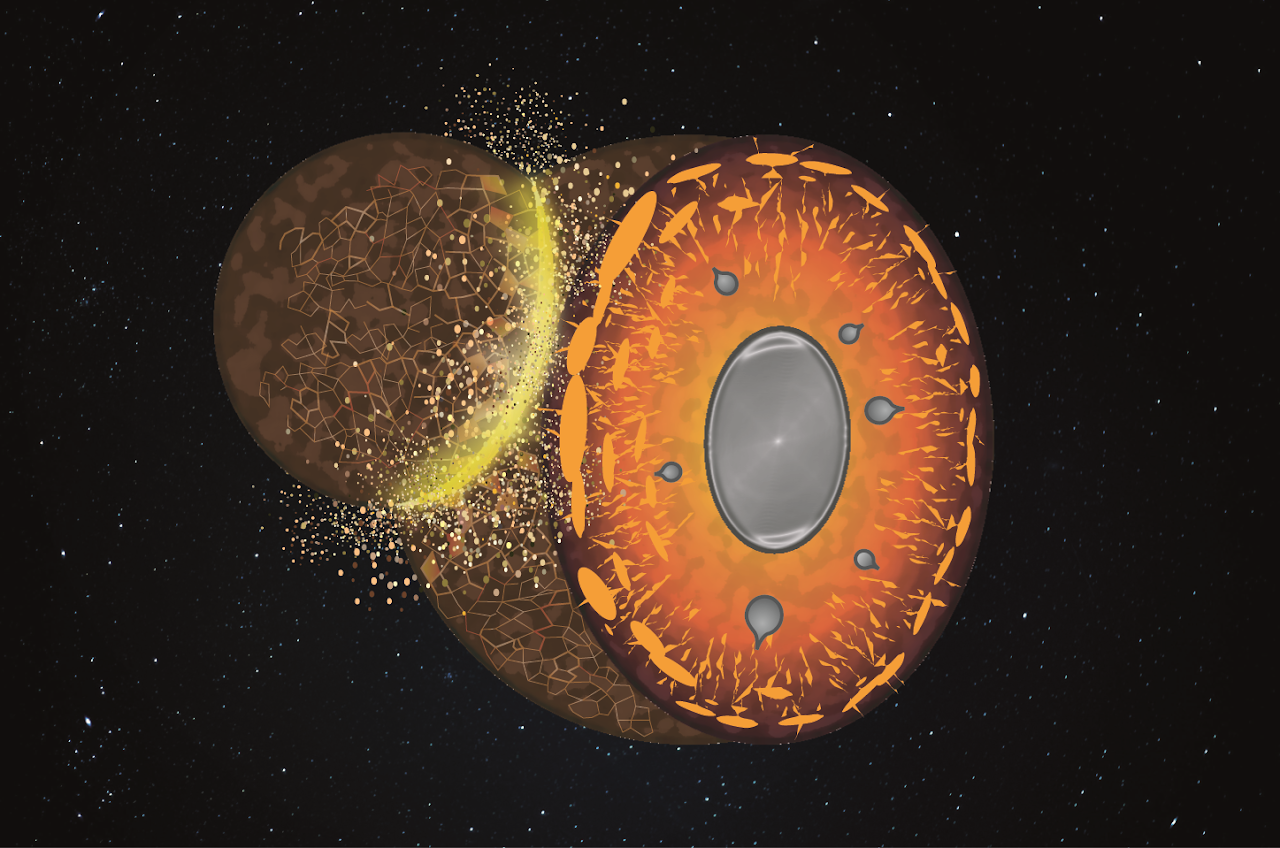
Says EPL staff scientist Anat Shahar, “Understanding the introduction, transport, and storage of volatile elements in the interiors of terrestrial-like planets is profoundly linked to the nature of habitability and the sustainment of life”
Volatile elements like carbon, hydrogen, oxygen, nitrogen, and sulfur are a cornerstone of planetary habitability yet it is unclear how the terrestrial planets obtained them and what their original budgets were. These elements are often mobile and readily exchange among reservoirs ranging from interstellar to sub-mm in scale. At EPL we are seeking answers to the many questions relating to the inventories of volatile elements and how they are affected by a range of processes during planet formation.
How do planets get volatile elements to being with?
The history of volatile elements starts in a star’s protoplanetary disk and continues through the planetesimals that collide to form a planet. Extensive heating and melting of objects throughout the often-violent growth process from planetesimals to planets assures that atmospheres will have formed through extensive interior degassing, with multiple episodes of atmosphere formation possible.
What happens to the volatile elements after a planet forms?
Planetary-scale geologic processes then partition volatile elements between the interior and surface, and these processes depend on the differentiation history of the object, the initial inventory of volatile elements, and the timing of their delivery to the planet.
Much of the initial partitioning of volatile elements between the atmosphere and the planetary interior occurs during a planet's very early history—as giant impacts drive extensive melting and the development of magma oceans. As the magma ocean crystallizes, some volatile elements will become a part of the crystallizing solids whereas others will be released into the atmosphere as gasses. How this partitioning occurs will change as the pressures and temperatures of crystallization change. In addition, the changing oxidation state in a solidifying magma ocean can impact how much of the volatile inventory can be incorporated into the crystalizing interior over time. These features of the magma ocean—pressure, temperature, and oxidation—could have major consequences for the composition of a newly-formed planet's first atmosphere.
3) What phases hold and transport volatile elements in deep Earth cycling?
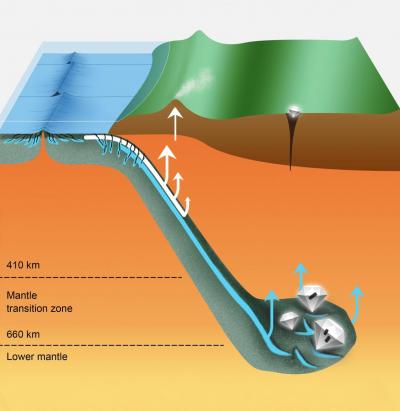
Says EPL Staff Scientist Michael Walter, “The key to understanding geochemical mass transfer in Earth is in tracing the movement of volatile-rich melts and fluids in the mantle and crust – melts that often leave an indelible chemical stain on the rocks they pass through”.
Once Earth was fully formed and volatile element budgets for the whole planet effectively established, the next question is “how are volatile elements partitioned between its major reservoirs: the core, mantle, lithosphere, and exosphere (hydrosphere+atmosphere)?” How do they cycle through these reservoirs over the age of the planet? In what solid and melts phases are volatile elements stored and transported in Earth’s interior? Does deep mantle cycling of volatile elements moderate their abundances in the exosphere over geological time? What role does the core play as a volatile element holding tank and does it exchange volatile elements with the mantle? At EPL we are using a range of petrological, experimental, geochemical, and theoretical approaches to investigate these questions.
Do volatile elements ride subducting oceanic crust into the deep Earth?
One of the key drivers of the deep volatile cycle is subduction. Interaction between the exosphere leads to the addition of hydrogen, carbon, sulfur, and nitrogen to oceanic crust and lithospheric mantle rocks. When surface plates plunge into the mantle at subduction zones they take a cargo of volatile elements with them.
Work at the Department of Terrestrial Magnetism was the first to conclusively show that sediments deposited on down going oceanic plates make it to the source depths of the arc volcanoes associated with plate subduction when 10Be—a radioactive isotope of beryllium that is made by interaction with energetic cosmic rays in the upper atmosphere—was found dissolved in the lavas erupted in these volcanoes.
Deeper in the Earth, we probe deep volatile cycles directly by studying diamonds and their mineral inclusions that form as a result of devolatilization processes when the subducting plates reach the transition zone and shallow lower mantle (~400—800 km). We are also using high pressure-temperature experiments to investigate which phases are the most likely carriers of volatile elements in the deep mantle, and in particular, we would like to know whether and the amount of volatile elements can be transported via subduction all the way to the core?
For example, weCarnegie’s Yanhao Lin and Michael Walterrecently discovered that the dense high-pressure forms of the common crustal mineral quartz, stishovite, and CaCl2-type structured phases, can contain at least 3 wt% water at transition zone and shallow lower mantle conditions [Lin et al PNAS, 2020], and because they are stable at mantle temperatures these phases can potentially transport water in subducted oceanic crust all the way to the core-mantle boundary. We are currently using laser-heated diamond anvil cell experiments coupled with first-principles molecular dynamics calculations to unravel just how water dissolves in these and other important phases.
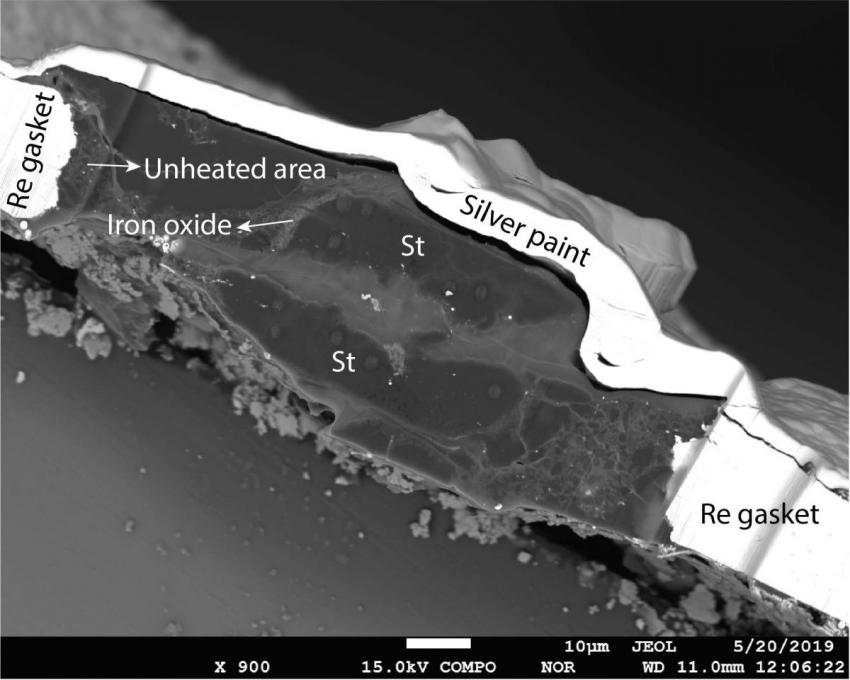
What kinds of volatile-rich melts could form in the deep mantle?
Phases rich in volatile elements tend to break down at high temperatures and release their volatile elements, which often instigates partial melting. Melts are hugely effective agents of volatile element transport in Earth’s interior. Understanding their compositional variation, densities, and physical properties are key to reconstructing deep volatile cycles. We are currently using experiments at transition zone and lower mantle pressures in the multi-anvil device to understand the kinds of volatile-rich melts that form in these regions and their physical properties, and how they relate to diamond formation and volatile storage and migration in the mantle.
4) How do volatile elements dissolve in silicate melts?
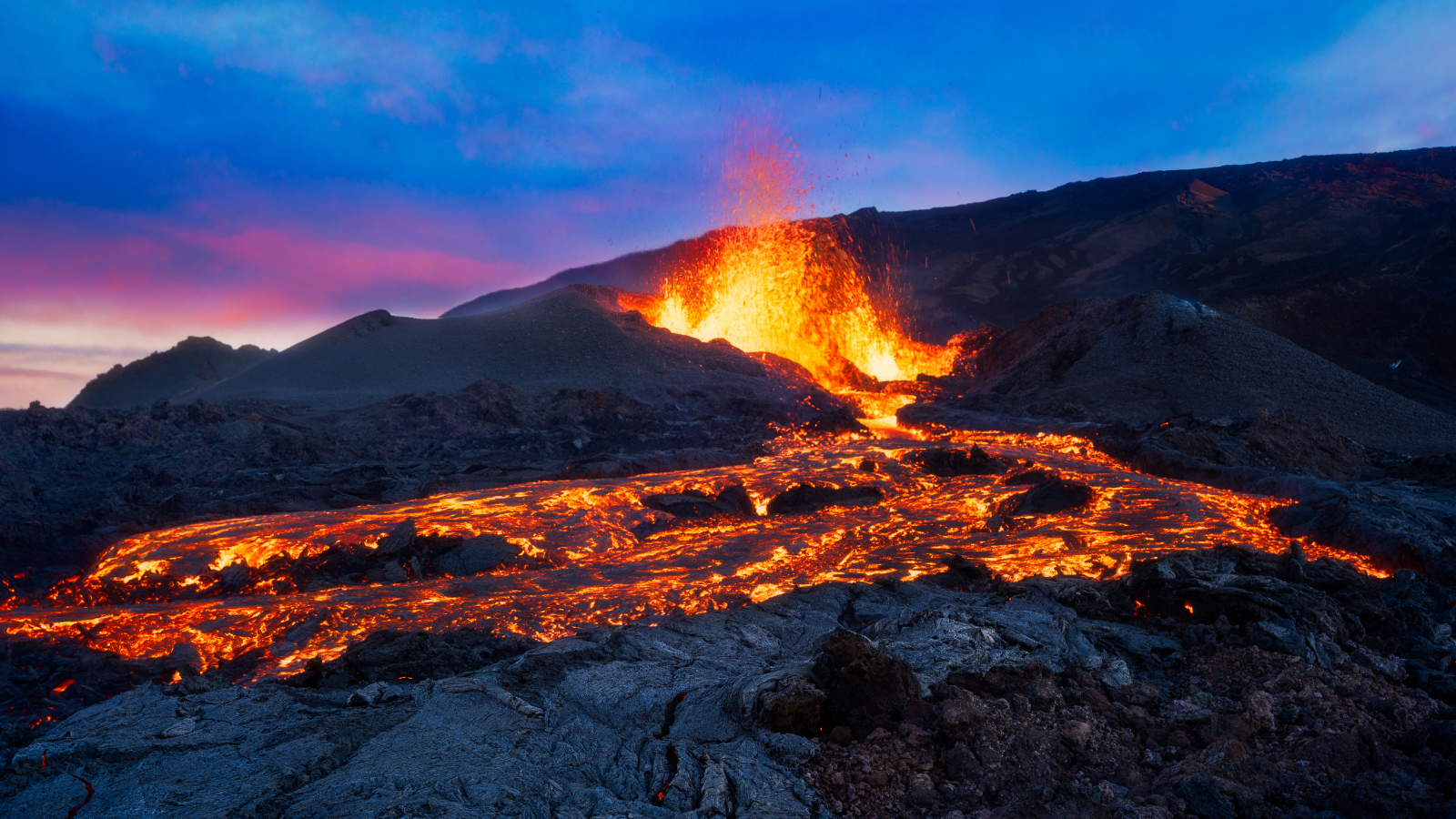
Understanding how the deep Earth communicates with the Earth’s atmosphere and hydrosphere requires knowledge of the processes that connect the deep Earth to the surface, processes that invariably involve partial melting of mantle and crustal rocks.
How silicate melts are generated and migrate is strongly controlled by the very powerful role of even trace amounts of volatile elements. This is important because volatile-laden silicate melts are the primary means by which matter and energy move through the mantle and crust to Earth’s surface. Volatile-element-rich melts also are responsible for the most dangerous forms of explosive volcanism.
We know that the physical properties of silicate melts—like their density, viscosity, resistivity, and molecular diffusivity—are strongly controlled by the molecular structure of the melts themselves. Even small amounts of volatile elements can significantly modify melt structure and change these properties, often by many orders of magnitude. These changes are far in excess of what the variables of pressure or temperature typically provide. Nevertheless, our understanding of how volatile elements interact with melt structure is largely phenomenological such that a detailed understanding of volatile-melt interactions remains poorly understood. Ultimately, a complete understanding of the molecular structure of volatile-bearing silicate melts is essential.
Does water change silicate melt structure?
Recently, using solid-state NMR we found that water breaks down silicate melt structure far beyond what had been previously assumed. Water amongst the many volatile compounds is apparently the most powerful melt structure modifier, but we have also identified modifications to melt structure derived from carbon and nitrogen as well.
How these interactions change as a function of pressure and temperature is an open question. We do not understand the molecular-level interactions of volatile species with silicate melts nearly well enough to accurately model deep Earth volatile cycling. Detailed studies using advanced molecular spectroscopic techniques such as NMR, Raman, and X-ray absorption techniques coupled with first-principles calculations are advancing our understanding of how volatile elements affect the melt structure at the atomic level.
5) How do we relate seismology to interior composition?
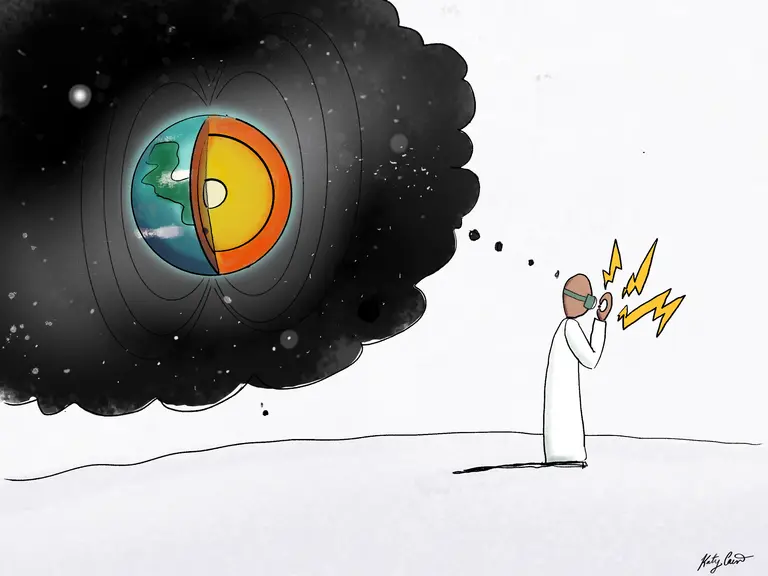
“The ability to make these measurements is crucial to developing reliable models of the internal structure of super-Earths up to eight times our planet’s mass,” Staff Scientist Yingwei Fei stated in a recent press release. “These results will make a profound impact on our ability to interpret observational data.”
Generally speaking, the Earth consists of a silicate mantle, a liquid iron-dominated outer core, and a solid iron-dominated inner core. We know this thanks to seismologists, who use earthquake waves like ultrasound to image the interior structures of our planet.
Research in experimental petrology and mineral physics is a key tool that allows us to determine the inner structure of our planet in more detail. Using high pressure and temperature experimentation, we can relate the chemistry and mineralogy to what we see in observed seismic profiles— from the surface to the center of the core.
What causes seismic discontinuities?
For example, for decades we have observed seismic discontinuities at 410-km and 660-km depth in the mantle. We think these discontinuities come from the olivine-wadsleyite polymorphic phase transition (410-km) and the transition where ringwoodite decomposes to a bridgmanite + ferropericlase assemblage (660-km).
High-pressure devices like the multi-anvil press allow us to mimic the environment of the inner Earth and precisely determine what is happening at these transition boundaries. By comparing the lab-based transition pressure of these minerals to the seismic discontinuity depth, we can confirm the mineralogy and chemical behavior of the deep Earth.
We also use the seismic profiles—the changes of velocity or density as a function of depth—to deduce the composition of the mantle and core. Based on geochemical constraints provided by surface rocks—typically melts from the mantle or xenoliths of the uppermost mantle— we built a series of compositional models for the interior. To test the models, we select minerals that we might find in the mantle and core and experimentally subject them to the pressure and temperature changes they would experience deep inside of our planet. We measure their densities and sound velocities in high pressure and temperature experiments and compare the results to the models we created.
Does a planet’s interior-dynamics affect habitability?
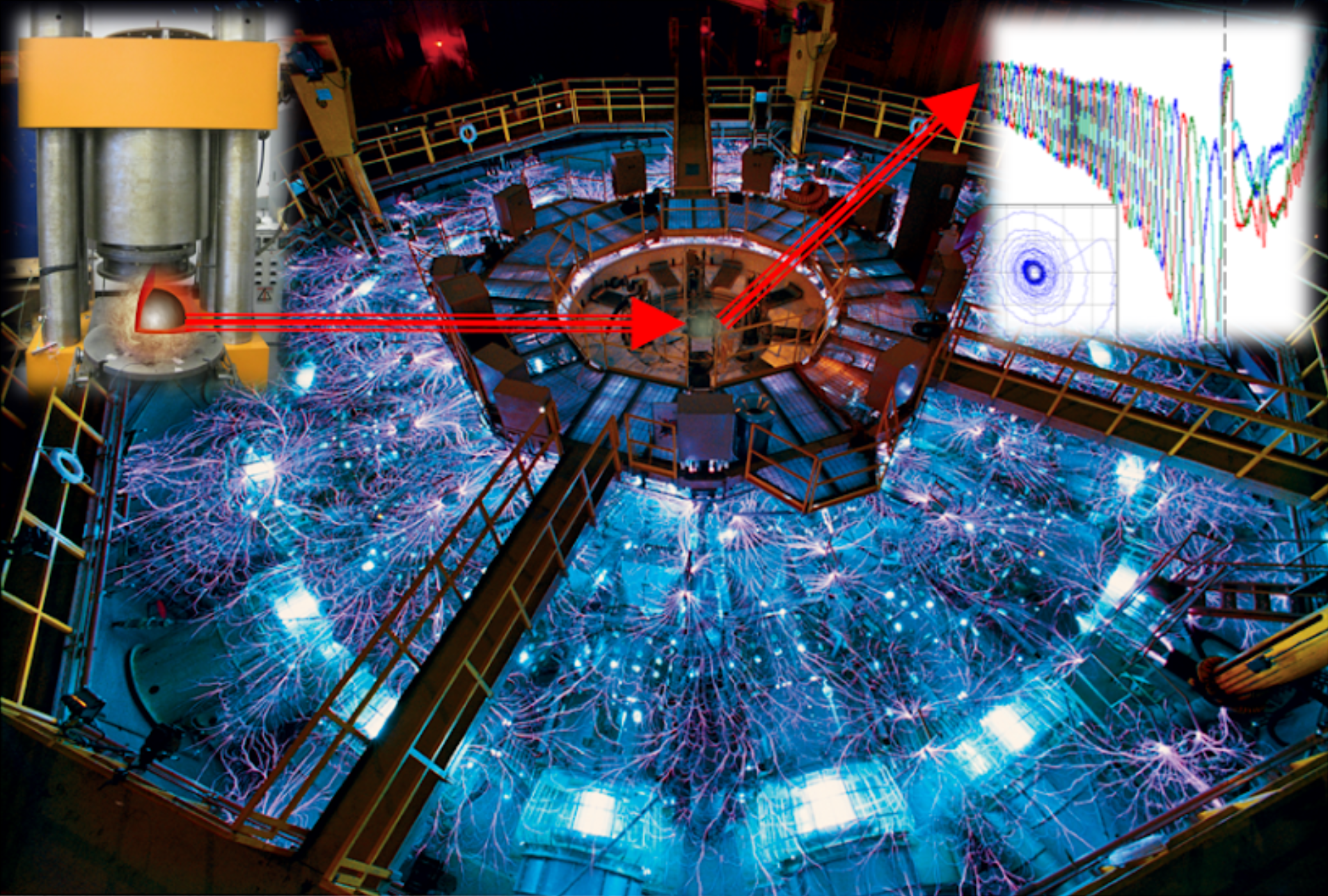
We are using a similar approach to understand the internal structure and mineralogy of super-Earths—exoplanets many times the mass of Earth but with Earth-like features. Because pressures within super-Earth sized mantles may exceed 1400 GPa (our planet’s inner core is only around 330 GPa), we use Sandia’s Z Pulsed Power Facility to observe density and melting of mantle bridgmanite as the mineral is shocked along a pressure-temperature path (“Hugoniot”) to extremes in pressure (> 1200 GPa) and temperature (>7000K) (pictured above). The result can be used to construct a mantle density profile for a model super-Earth and infer a possible mantle/core fraction based on the observed mass-radius relation of the super-Earths.
6) How is heat transported in the deep mantle?
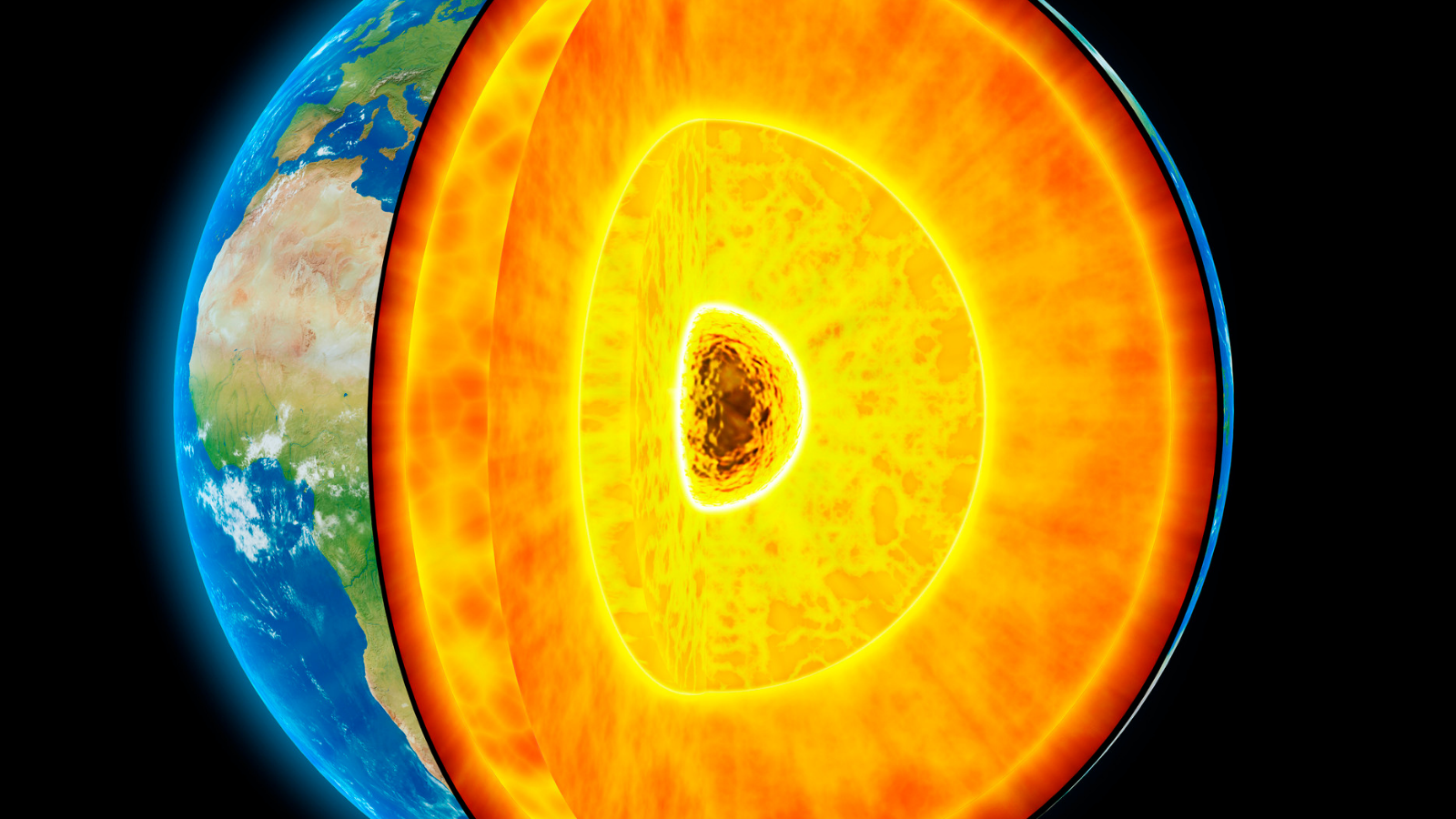
The most basic features of heat transport from the top of the core to the top of the mantle are well understood: the highly conductive liquid outer core delivers as much heat to the base of the mantle as the mantle can absorb. The mantle, in turn, absorbs heat by conduction through its deepest layer, heat that is then transported by convection in all other regions of the mantle and ultimately to the lithosphere where it is released through volcanism and conduction.
The amount of heat leaving the core is a big deal because this feature largely controls the timing of inner core crystallization and generation of the magnetic field. The major unknowns that today’s researchers are investigating are focused on the lowermost layer of the mantle where conduction is dominant. What is the thickness of this layer? What is the rate of heat flow through this layer? What is the temperature difference across this layer? What is the temperature profile of the core?
We have developed a range of experimental and theoretical tools to interrogate these questions that include ultra-fast pulse-probe techniques to measure thermal conductivity in metals and silicate and latent-heat melting probes.
How does heat flow across the core-mantle boundary?
For example, by combining an ultrafast time-domain thermoreflectance (TDTR) technique with the diamond anvil cell (DAC) we directly measured the thermal conductivities of two main components of our planet’s core—Fe and Fe–Si alloys. What we found suggests a lower heat flow across the core–mantle boundary than previously expected. This means less thermal energy than we originally thought is needed to operate the geodynamo that creates our planet’s protective magnetic field. It also suggests an inner core age that could be older than two billion-years, meaning the core may have formed earlier in our planet’s history than we thought [Hsieh et al, 2020].
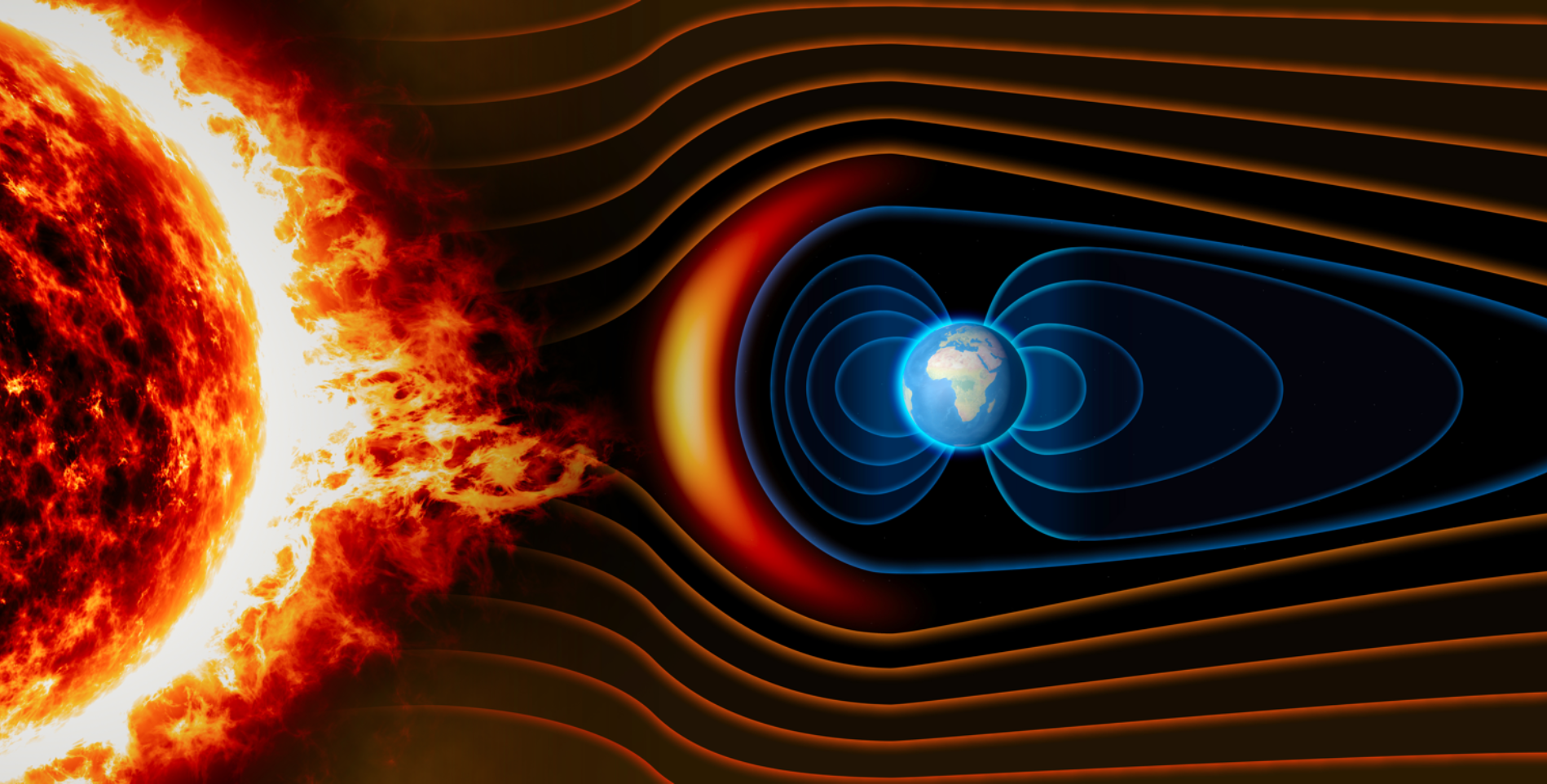
In a 2020 press release about the study's findings, Staff Scientist Alexander Goncharov stated, “The less thermally conductive the core material is, the lower the threshold needed to generate the geodynamo. With a low enough threshold, the heat flux out of the core could be driven entirely by the thermal conduction, with no need for the additional movement of material to make it work.”
What is the actual temperature difference between the mantle and core?
At the same time, we used a combination of continuous and pulsed laser heating in a diamond anvil cell to measure the lattice thermal conductivity of lower mantle minerals. We are able to use this information to constrain the estimated the thickness of the conduction-dominant lowermost layer to be between 30 to 400 km [Geballe et al. 2020]. Now we are working on establishing the temperature difference between the mantle and core in ongoing experiments using a new latent-heat-based experimental technique [Geballe et al. 2021].
The diamond anvil press is one of our most important tools. Scientists use it to generate pressures like what we might find in the deep Earth. We then use lasers to heat the samples to extreme temperatures. This allows us to study the interior chemistry of our planet in a laboratory setting.
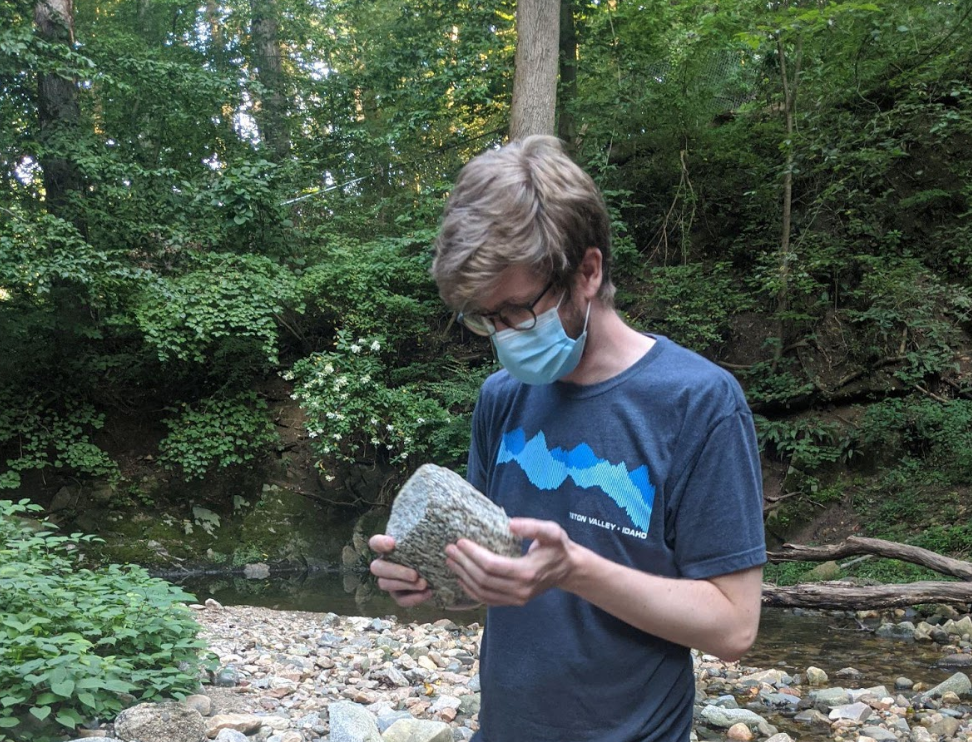