Where were you on the night of the 3rd...4.5 billion years ago?
You may not remember what you were up to last month, but rocks have billion-year memories. The chemical signatures of rock and space dust hold clues to the deep histories of our planet, Solar System, and life itself—if you know where to look. This month, we're highlighting the chemical detectives on campus who know exactly how to get these rocks to talk: the Carnegie Science Earth and Planets Laboratory’s world-class team of geochemists and cosmochemists.
These scientists use specialized equipment to precisely measure the chemical and isotopic compositions in minuscule samples of rocks, space dust, solar wind, and more—sometimes down to counting individual atoms. Then they fit each new isotopic marker or molecule into the complex chemical equation that makes up the cosmos. Their goal? To create a timeline of the Earth and our Solar System's early development.
Our cosmochemists address the formation and early evolution of our Solar System, of meteorites and asteroids, and larger bodies like Mars, Mercury, and the Moon. Closer to home, our geochemists combine laboratory and field studies of modern and ancient rocks from diverse geographic areas to investigate the origin of Earth’s crust and the dynamic processes that exchange material between surface and interior.
Below, the geochemists and cosmochemists of EPL discuss the top questions in the field:
- How did the conditions in the early Solar System determine the composition of planets?
- Where did Earth's water come from?
- What is the origin of organic molecules in space and were they the building blocks of life on Earth?
- How does plate tectonics control the deep Earth recycling of carbon, water, and other essential elements?
- How did the first continents form and then evolve to their present age structure?
How did conditions in the early Solar System determine the composition of planets?
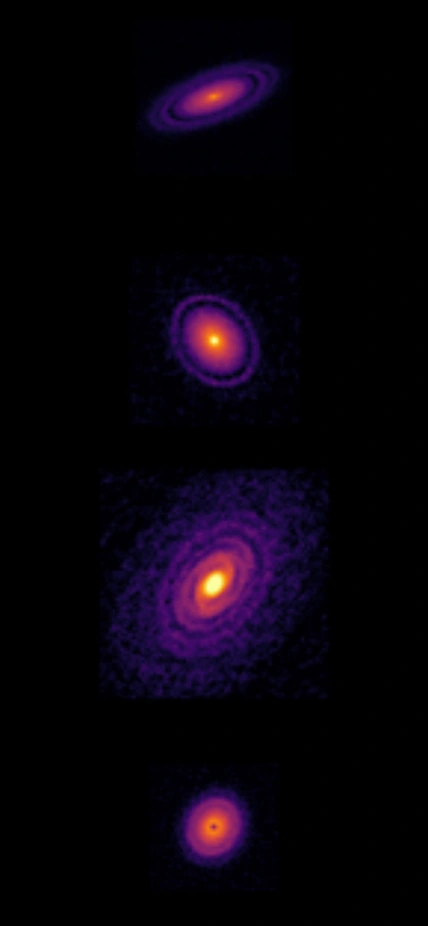
Images of circumstellar disks obtained with the Atacama Large Millimeter Array (ALMA) radio telescope. Credit: ALMA, ESO/NAOJ/NRAO.
Our Solar System started as a diffuse cloud of dust and gas at a density of a few hundred molecules per cubic centimeter and a temperature of about 10-15 degrees above absolute zero. Something caused that cloud to collapse, either the gravity of its own material or perhaps it was given a “push” by the shock wave from an exploding nearby star in the galaxy. As the cloud collapsed, gravity took over to gather most of the mass of the cloud into the central star, the Sun in our case. Because the molecular cloud was slowly rotating before the collapse, angular momentum caused a small percentage of the collapsing cloud to form a flat disk of material around the Sun.
The alignment of the planets along a single plane, the ecliptic plane, is good evidence of their formation from a disk of this nature.
Close to the central star, the disks were very hot—hot enough that most of the material was vaporized. As the disk eventually started to cool down, mineral grains began to condense, starting with those containing calcium, aluminum, and titanium that condense at the highest temperatures. Accumulations of these refractory grains, called calcium-aluminum inclusions (CAI) are found in some primitive meteorites and provide ages of 4.568 billion years—setting the start time of the planets in our Solar System.
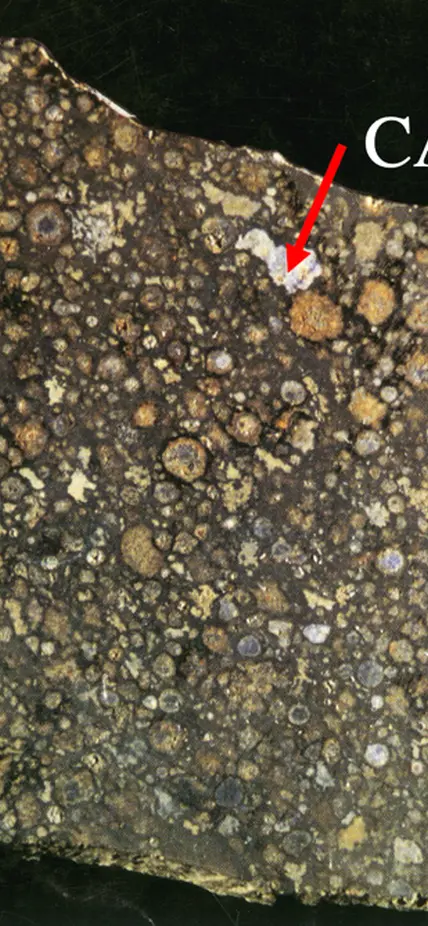
Photograph of a section of a chondritic meteorite. This type of meteorite is essentially sediment composed of a variety of different mineral grains that came together in the early history of the Solar System. They are composed of CAIs, but mostly the round objects called chondrules that give this group of meteorites their name. Chondrules are quickly cooled droplets of silicate melt. Their mechanism of formation is the topic of work by Conel Alexander.
On further cooling, the major silicates—olivine, and pyroxene—began to condense. Further away from the Sun, where temperatures were colder, various ices such as water and carbon monoxide also could condense. The boundary in the present asteroid belt between the relatively dry and rocky inner planets and the huge gaseous and icy outer planets can be thought of as a “snow line” where ices condensed only outside the snow line. The snow line concept provides a first-order explanation for why we have rocky and icy planets.
Digging deeper, our analyses of the neodymium and chromium isotopic composition of different types of meteorites along with rocks from Earth, Mars, and the Moon provided an early indication that the group of meteorites believed to come from further out in the Solar System—the carbonaceous chondrites—are made up of a different mixture of Solar System components than Earth and groups of meteorites thought to originate from the inner Solar System.
Other studies have confirmed and extended this distinction to arrive at the suggestion that Jupiter may have formed very early in the Solar System, opening up a gap in the disk, isolating the inner from the outer Solar System. If so, this could explain why the terrestrial planets are depleted not only in water but in other moderately volatile elements like the alkali metals and lead. Modeling the composition of Earth based on the various components found in meteorites can provide a reasonable match to Earth compositions on the assumption that Earth is made up primarily of the more refractory components in meteorites (CAIs and chondrules) with only a smaller role for the more volatile-rich matrix component seen in carbonaceous chondrites.
This model provides additional support for the idea that the early formation of Jupiter may have cut off the inner Solar System from the more ice-rich objects formed in the outer Solar System, leading them to be relatively deficient in water and other volatile components.
Where did Earth's water come from?
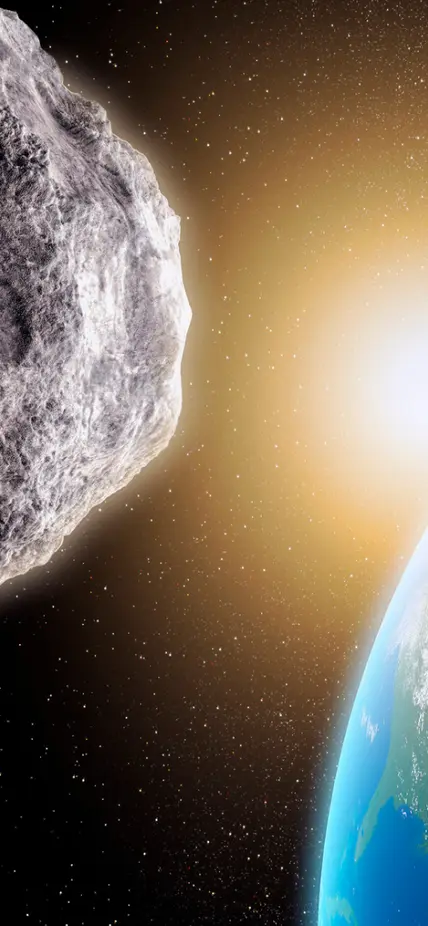
The origin of Earth’s water is an open question and quite controversial. It really is part of a larger question about how Earth got its very volatile elements that include not just the hydrogen in water, but also carbon, nitrogen, and the noble gases (helium, neon, argon, krypton, and xenon) amongst others. Ultimately, any complete explanation must be able to reproduce both their elemental abundances and their isotopic compositions. It must also be compatible with what we know about how and on what timescales planetary systems form from the disk of gas and dust (protoplanetary disk) that surrounds a young star. In the case of the Solar System, the protoplanetary disk around the young Sun is generally referred to as the Solar Nebula.
Currently, the most favored explanation for where the Earth got its water is that it acquired it from water-rich objects (planetesimals) that made up a few percent of its building blocks. These water-rich planetesimals would have been either comets or asteroids. From what we know about comets, they are not a good match to either the elemental or isotopic composition of the Earth. It may also have been difficult to deliver enough comets to the Earth.
Water probably came from asteroids
At present, asteroids up to a few hundred kilometers across seem the most likely sources of most of Earth’s water, specifically the types of asteroid that dominate the outer asteroid belt between Mars and Jupiter. We have samples of some of these asteroids in the form of CM and CI carbonaceous chondrite meteorites. When they formed, these asteroids/meteorites contained water-ice, but radioactive heating caused the ice to melt and react with the anhydrous minerals to make clays. The meteorites also contain quite a bit of organic matter that contains hydrogen, carbon, and nitrogen – the hydrogen would have reacted to form water when the asteroids impacted the early Earth.
Noble gases even seem to be associated with the organics. The CM chondrites, in particular, give a reasonable fit to many aspects of Earth’s highly volatile elemental and isotopic compositions, although small amounts of cometary and Solar Nebula material produce an even better fit. Nevertheless, disagreements remain and there is much debate about whether these are due to some combination of (i) inaccuracies in estimates of the Earth’s volatile contents, (ii) storage of hydrogen and other volatiles in the Earth’s core, and (iii) loss of volatiles to space in the giant impacts that dominated the final stage of Earth’s formation.
Where do water-bearing asteroids come from?
If CM- and/or CI-like chondrites were the main sources of Earth’s water, where did they come from? It looks like they are representatives of a swarm of planetesimals that formed in the colder regions of the Solar Nebula beyond the orbit of Jupiter where water-ice-rich dust was plentiful, and they were scattered into the regions where Earth and the asteroid belt formed by a growing Jupiter.
What is the origin of organic molecules in space and were they the building blocks of life on Earth?
By Larry Nittler, George Cody, Conel Alexander, & Dionysis Foustoukos
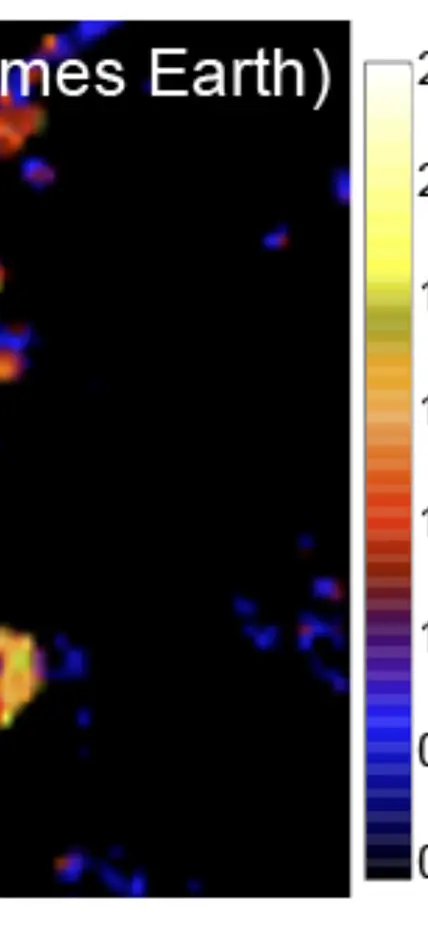
We know that complex organic molecules are abundant in space from telescopic observations of molecular clouds and protoplanetary disks, from spacecraft observations of comets and asteroids, and from laboratory analysis of meteorites and other extraterrestrial materials. Comets and asteroids delivered large amounts of such molecules, including life-essential amino acids, to the Earth as it was forming, perhaps providing the non-biological building blocks from which life was able to eventually emerge.
At the Earth and Planets Laboratory, Conel Alexander, George Cody, Dionysis Foustoukos, and Larry Nittler study organic material in meteorites and comet samples in order to learn how and where it formed and to better understand the original molecular inventory of Earth.
Primitive meteorites (those that come from asteroids that have experienced very little heating after their formation) contain up to several percent of their weight as organic carbon. Some of this is in the form of water-soluble molecules like amino acids, but most of it is in a more coal-like, complex material known as IOM (for insoluble organic matter).
George Cody uses the techniques of Nuclear Magnetic Resonance spectroscopy, synchrotron-based X-ray spectroscopy, and infrared spectroscopy to tease out the complicated molecular structure of meteorite IOM and infer possible formation mechanisms. His work has shown that the chemical nature of the IOM is consistent with formation in the Solar System from a chemical reaction series starting with the simple molecule formaldehyde, which is known to be present in the icy mantles of interstellar grains from which planetesimals ultimately accrete early in Solar System history. In a series of experimental studies, George Cody together with Dionysis Foustoukosalso probes how low levels of heating in asteroids modifies the molecular structure of IOM.
Conel Alexander and Dionysis Foustoukos study the average elemental and isotopic composition of organics in different types of meteorites. Interestingly, meteoritic organics, including IOM, show unusual isotopic characteristics; much of the material is highly enriched in the rare isotopes deuterium and nitrogen-15 (heavy forms of hydrogen and nitrogen, respectively). Such signatures can most easily be explained by chemical reactions occurring at very low temperatures and may point to a presolar origin of the organics (or their precursors) in the molecular cloud from which the Sun formed.
Furthermore, experimental studies have identified physicochemical processes that may control the evolution of these rare isotopes in altered IOM. Larry Nittler and Conel Alexander use high-resolution secondary ion mass spectrometry to look at very small-scale isotopic variations in meteoritic organics. Such work has found extreme variability in the isotopic composition of the organic matter on micrometer scales (figure). By looking at both the bulk and micro-scale chemical variations within and between different meteorites, the EPL researchers hope to disentangle the effects that occurred on the meteorites’ parent asteroids (e.g. heating and interactions with liquid water) from the original properties of the organic matter to fully understand its origin, how it is modified during planet formation, and what eventual role it may have in the origin of life on Earth.
How does plate tectonics control the deep Earth recycling of carbon, water, and other essential elements?
By Steve Shirey
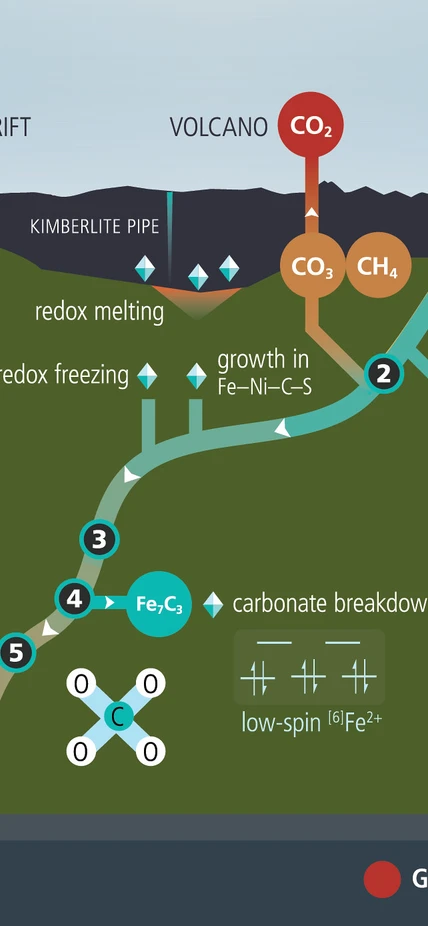
A simplified diagram of the reactions that occur during the deep-Earth carbon cycle. Image credit: Deep Carbon Observatory
Plate tectonics was a revolutionary idea that changed Earth Science when it was introduced in the 1960s. The theory holds that the uppermost 100 to 250 km of Earth’s ~6400 km radius is made up of rigid plates that move around, crack, and deform. This movement has been powered since the Earth formed 4.57 billion years ago by the inexorable heat engine of its mantle and core. This heat engine moves hot but solid mantle rock from the boundary zone at the core to crystallize as tectonic plates at the surface, thereby removing the heat coming from three basic sources: heat residual from planetary accretion, heat currently produced by inner core crystallization, and heat currently produced by radioactive decay in the mantle. The main consequence is for new lithospheric plates to be created at ocean ridges and to sink below the surface back into the mobile mantle at bands or zones of descending seismicity known as subduction zones. This process is known as subduction.
A fascinating aspect of subduction is the capacity for sinking lithospheric plates to take elements such as hydrogen, carbon, nitrogen, oxygen, boron, and sulfur, and compounds such as water, carbonate, sulfate, and methane from the biosphere, hydrosphere, and atmosphere in their constituent minerals and thereby carry them into mantle. Since these elements were originally exsolved from the mantle shortly after the planet formed, we call this process mantle recycling.
Three mantle recycling pathways
We can think of mantle recycling as operating on three identifiable geologic pathways that are often distinct in their mineralogical and petrological characteristics but also can overlap.
-
The first mantle recycling pathway is from the near-surface down 300 km. It involves dehydration of the plate, transfer of fluids into the mantle wedge, production of melt in the mantle wedge, and creation of island arcs and their associated active volcanism. While most warm plates follow this pathway, other plates are too cold to follow it.
-
This permits a second mantle recycling pathway to operate at depths from 350 to ~700 km whereby material is carried past the arc and wedge into Earth’s mantle transition zone and occasionally deeper into the top of the lower mantle. This pathway involves the recrystallization of dense hydrous carrier minerals that were able to form when the colder plate did not dehydrate and the evolution of fluids.
-
The third mantle recycling pathway is obscure because it cannot be easily related to current plate tectonic patterns. This mantle recycling pattern is evident in the background of mantle chemical variability that was created by the summed-up effects of the first two pathways operating over billions of years and other, earlier forms of recycling not known to be directly related to plate tectonics.
EPL mineralogists, petrologists, and geochemists have been working on the shallow recycling process that drives arc volcanism since the very advent of plate tectonics.
Because the site of arc volcanoes is also the site of tremendous seismicity, there has always been a natural partnership between geophysicists and geochemists/petrologists that is ideal for the kind of intellectual cross-fertilization for which the Carnegie Institution for Science is famous.
Arc lavas are the wettest magmas on Earth and early focus was on the role of water in modifying the melting relations of the mantle through experimental petrology and verifying the direct link between sediments carried down by the plate during subduction and sedimentary geochemical signatures using cosmogenic radionuclides that only stick to sediments. The arrival of secondary ion mass spectrometers on campus gave EPL geochemists the chance to measure the water content directly in a host of volcanic glasses and tiny pockets of glass (melt) hosted in phenocryst minerals known as melt inclusions. Now it was possible to follow the recycled water directly.
This capability became essential during the decade of research of the Deep Carbon Observatory where EPL scientists hatched the ambitious plan to quantify the gaseous output of Earth’s volcanoes with direct gas measurements and compare it to the glass volatile content.
How did the first continents form and then evolve to their present age structure?
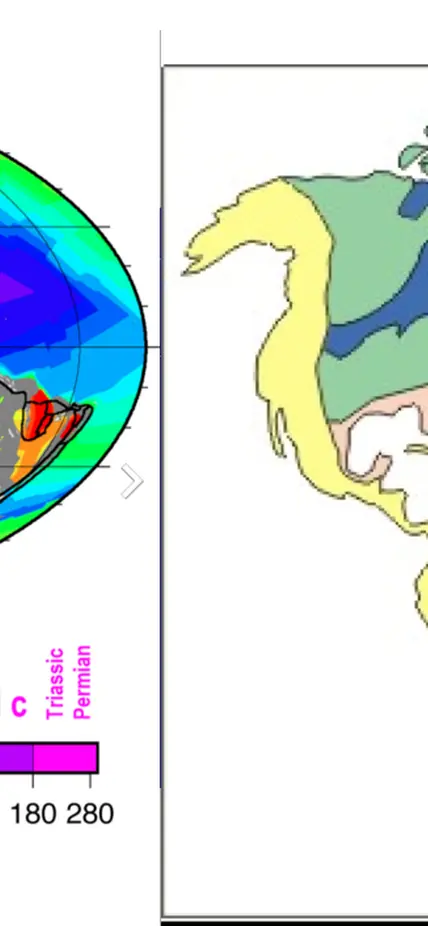
Earth is unique amongst the terrestrial planets in having two main elevations for its surface—ocean basins that are on average, about 4 km below sea level and continents that for the most part are less than a kilometer above sea level.
Why is the continental crust older than oceanic crust?
The other big difference between oceanic crust and continental crust is that the former is everywhere less than 300 million years old, whereas some sections of continental crust are as old as 4.3 billion years.
The youth of oceanic crust is because it is continually being made along mid-ocean ridges and destroyed when it plunges back into Earth’s interior along subduction zones. When continents collide, however, they tend to buckle and thicken rather than sink into Earth’s interior—for example, the Himalayan Mountains were caused by the collision of India and Asia. The different behavior reflects the relatively low density of continental crust, which is on average the composition of andesite (a rock with about 60% SiO2 and a density of 2.86 g/cc), compared to oceanic crust, which is made of the denser basalt (a rock with about 45% SiO2 and a density of 3 g/cc.)
An additional contributor to the long-term survival of continental crust is that old sections of continents are underlain by 100-200 km thick sections of mantle, which are the residue of past partial melt extraction—perhaps the melts that now make up the continental crust. The partial melt leaves behind mantle that is slightly less dense and much stronger due to the removal of water with the melt. With time, these continental “keels” become colder than the surrounding mantle, which makes them the main host of diamonds because the conversion from diamond to graphite is enhanced at higher temperatures.
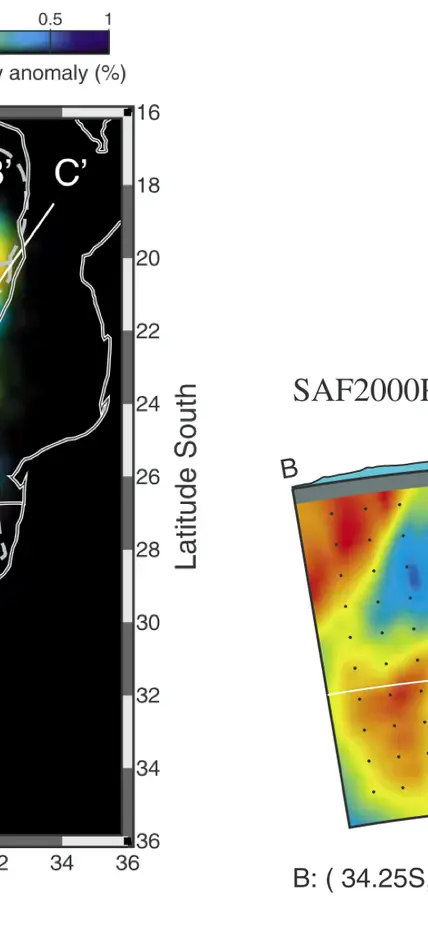
Seismic velocities beneath southern Africa. Blue colors denote high seismic velocities indicative of cold temperatures whereas red is lower velocity, higher temperature, material. The coldest regions, extending to 200-300 km depth in the cross section on the right are centered beneath the oldest crustal section in this area, the Kaapvaal Craton that dates back to 3.6 billion years. Figures from James et al., G3, 2004.
How does the Earth’s crust form?
The mechanism of the formation of oceanic crust is reasonably well understood. As plates pull apart, the underlying mantle rises into the opening rift. The decreasing pressure as the mantle rises lowers its melting point leading to the production of basalt, the most common melt of mantle material.
The higher silica rocks of the continental crust, however, cannot be made directly by melting of dry mantle rocks.
Instead, their genesis must involve water and possibly the melting of basaltic rocks that have been altered by water. Both potential source components of the “granitic” rocks of the continents are found in subduction zones, where basaltic oceanic crust altered by interaction with ocean water plunge back into the mantle. The water they carry into the mantle instigates the type of melting that produces the volcanos found all around the Pacific “ring of fire” from southern Chile through the Cascades to Alaska and the down the western Pacific from Kamchatka to Indonesia. Whether this is where the first continents were created is not yet settled, in part because of the continuing debate over when plate tectonics and the subduction of oceanic crust began.
When did subduction start?
Our work has focused on the oldest sections of the continent, from the 3.6-billion-year-old rocks of southern Africa to the over 4-billion-year-old rocks in two areas of northern Canada. Though each area records its own unique path to creating the ancient crust, a common theme seems to be the creation first of a thick pile of basalt, for example like the current crust of Iceland. That basaltic crust then gets hydrated by interacting with surface water.
Our work in the old rocks exposed along the eastern shore of Hudson’s Bay provides evidence of large bodies, lakes, or perhaps oceans, of water on Earth’s surface as long ago as 4.28 billion years. As the basalt pile thickens, the base is pushed to greater depths where the combination of water and higher temperatures causes the basalt to melt, making the silica-rich rocks typical of continental crust. Once this kernel of continental crust is created, then subduction of oceanic crust beneath this proto-continent can cause the type of metamorphism and volcanism that is still creating continental crust today.
In the Northwest Territories of Canada, this transition is recorded by the 4-billion-year-old Acasta terrane to which a large section of new continent was added about 3.5 billion years ago—likely through plate convergence. This area provides a good early example of the growth of continental crust through the accretion of new to older sections of crust, forming the patchwork of ages seen in the continents.
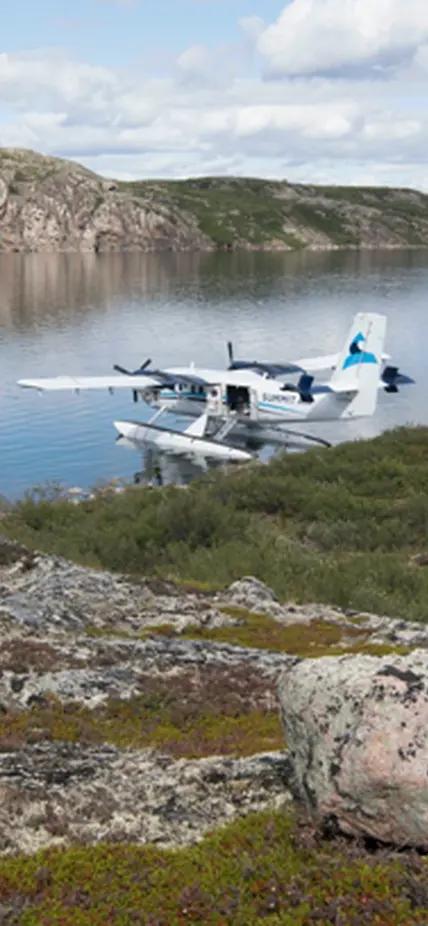
Field party in the 3.5-billion-year-old rocks along Point Lake in the Northwest Territories of central Canada. Photo by Matt Scott.
Reading this record of the creation of Earth’s first crust is complicated by the long history of metamorphism experienced by any old terrane due to the continuing geologic activity on our planet. Modern geochronological techniques—many of which were developed here at the Carnegie Institution for Science Earth and Planets Laboratory—now provide the ability to see through at least some of these later events to give a clearer picture of the processes involved in creating Earth’s first continental crust.